Calculating Riverbank Erosion, Sediment, and Nutrient Loading Rates using LiDAR
- Emma Hayes
- Apr 16, 2021
- 7 min read
Riverbank erosion varies in its contribution to suspended sediment loads due to different contributing factors including climate, topography, geology, soils, and land usage (Thoma et al., 2005). Agricultural practises are suspected as the major contributing source to pollution for many waterways. As phosphorus is adsorbed to soil particles, this in combination with suspended sediment introduced through riverbank erosion, contributes to waterway degradation through eutrophication and sedimentation (Sharpley et al., 2003). As the sources of sediment and phosphorus from agricultural systems are often from both field-based soil erosion and riverbank erosion, it can be difficult to determine the proportions delivered from these two independent sources. It is vital for the cost-effective introduction of management techniques for improved water quality that the dominant contributing source is known (Thoma et al., 2005).
To research the effects of sediment and nutrient components from agriculture on water quality, the cross-border Blackwater catchment in Counties Armagh, Tyrone, and Monaghan has been used for research. The Blackwater drains an area of 1480 km2 and has 90% land use categorised as agricultural, focused on intensive sheep, beef, and dairy farms (Bastola et al., 2011). Given the long-term over application rates of slurry and fertilisers across the island of Ireland following the post-war expansion and intensification of the farming industry, soils suffer from issues of legacy accumulations of nutrients, particularly phosphorus (Cassidy et al., 2019). With the role that phosphorus plays in contributing to eutrophication occurrence in waterways, reducing the soil’s content of phosphorus and the potential losses of phosphorus through mechanisms such as soil erosion, runoff, or riverbank erosion is vital to improve water quality.
Previous research conducted at the Blackwater study field sites (1:1,000-1:3,000 scale) aimed to identify and quantify the presence of nutrient hotspots through precision agriculture and gridded soil sampling. This allowed areas that have high nutrient index values to be located within each field and determine areas likely to be contributing increased nutrient loads through soil erosion or runoff. Given that these study sites are located within an agricultural catchment with each site having a river within its landscape, there is potential for nutrient and sediment losses through riverbank erosion. Recent research has focused on using fieldwork and desktop-based analysis in GIS to quantify the potential nutrient and sediment loading rates at each site through using LiDAR (Light Detection and Ranging) to identify areas of riverbank erosion. An example of one of these erosion areas commonly present in the study sites is shown in Figure 1.
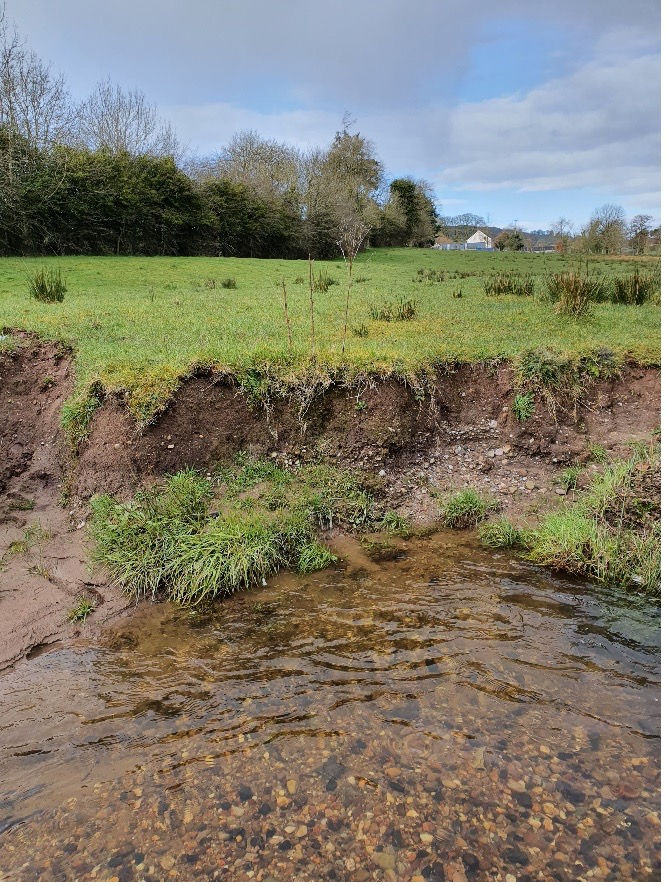
LiDAR offers itself as a good means to quantify large areas and allows a movement away from traditional erosion studies by using remote-sensing techniques to identify areas undergoing erosion. Studies such as Rose and Basher (2011) have used LiDAR successfully in catchments when combined with historical aerial photography to calculate riverbank erosion and meander migration on a five-decade basis. However, the usage of LiDAR to LiDAR comparisons is a more accurate means to determine erosion rates and can be widely replicated across various spatial scales or locations. This allows these eroding areas to be targeted for management and allows the potential contributing areas and/or land uses leading to waterway pollution inputs to be determined.
A study by Thoma et al., (2005) used LiDAR data to quantify and determine the contribution of sediment and nutrients in rivers such as phosphorus from riverbank erosion. LiDAR flights were used to create uniform 1 m digital elevation models of bare earth through vegetation stripping and interpolation. Image differencing was performed in GIS to determine the volume change over time. Mass wasting rates were calculated by multiplying net volume change with averages of bulk density. Inputs of sediment were then calculated by converting the mass-wasting rates into sediment load. Nutrient loads were derived by multiplying the mass of eroded sediment by the concentration of extractable P that was present in the samples. This allowed the contribution of riverbank erosion to total suspended sediment and nutrient loads in rivers to be determined. Such a methodology was adapted and used for the Blackwater catchment at the four study sites under question.
During August 2020, several cores of riverbank material at each of the field sites were collected which represented typical riverbank strata for the field site under question. These underwent analysis for bulk density and total extractable Phosphorus (using the Olsen P methodology as the standard agronomic soil test for phosphorus in Northern Ireland). A freely available 2014 LiDAR 1 m DTM (digital terrain model) dataset on the OpenDataNI website covered the extent of the field sites in the Blackwater catchment giving a baseline date of imagery and riverbank position. The Centre for GIS and Geomatics within QUB flew drone flights across the field sites to produce a DSM (digital surface model) for July 2020. As this is a DSM, this contains vegetation returns and these must be removed to produce a DTM that represents bare earth elevation. Processing was performed in ArcPro to remove vegetation returns through the functions of imagery, pixel editor, and terrain filter to produce a DTM for each of the sites.
The two LiDAR datasets used for analysis covered a period of six years. Therefore, to calculate annual rates of erosion, sediment, and P loads, the volume change value calculated due to erosion was divided by six to give an average annual estimate of erosion. To calculate this average annual erosion rate, the sum of elevation differences between the two periods was divided by the spatial extent covered by each site’s buffered riverbank zones to give an average volume change per vertex (or rather each co-ordinate). The 2014 and 2020 LiDAR datasets were first clipped to the extent of the field sites under investigation before the function of the raster calculator function was used to produce an image differenced raster under the expression of; “2014 LiDAR Dataset – 2020 LiDAR dataset” as the difference in elevation values between the two scans. Under this particular expression, positive values represented areas of erosion, with negative values indicated areas of deposition. This was then clipped in size to the buffered riverbank zones of each site.
Whilst the study conducted by Thoma et al., (2005) was interested in the net volume change of either erosion or deposition on a much larger scale, this work focused on the issue surrounding riverbank erosion and its potential to release sediment and nutrients. As such, using the function of extract by attributes with an expression of where “value > 0”, any negative areas representing deposition were removed from the differencing rasters. These new rasters containing only positive values of erosion were used in the tool of zonal statistics to calculate the summed total of elevation differences at each vertex for each riverbank. This summed value was then divided by the spatial extent covered by the riverbank to give an average change in elevation per-vertex, which represents the average rate of erosion for the area under analysis.
To give an average annual erosion rate per year this value was divided by six. To calculate an average mass wasting rate this per annum average erosion rate was multiplied by the average bulk density. To calculate the average annual input of sediment to waterways for the entire riverbank, the average mass wasting rate was multiplied by the spatial extent of the riverbank zones. Finally, to calculate the average annual inputs of total extractable phosphorus due to riverbank erosion, this average input of sediment was multiplied by the average total extractable phosphorus concentration to determine average phosphorus loading rates.
The following Table 1 shows the results for the average annual rate of erosion, sediment, and total extractable phosphorus loading rates for each site. In this table, both riverbank values have been combined for each parameter to give an overall site value, however, this methodology allows each riverbank face to be analysed individually and infer if a particular riverbank face undergoes a greater rate of regression than another at a site level.

These results demonstrate the wide variability existing in erosion rates across four similar study sites within the Blackwater catchment. Site three in particular has concerning rates of erosion, with a calculated overall annual average erosion rate of close to 80 cm per year, it releases significant quantities of sediment and phosphorus into this waterway.
Interestingly soil sampling at this site showed largely optimum soil phosphorus levels, yet due to its high erosion rate, this site contributes the greatest losses of the four study sites of over 128 kg of sediment per year and releases 2.93 g of phosphorus per year, solely through riverbank erosion. This highlights the need to have wide-ranging information and research quantifying all potential losses of sediment and nutrients from agricultural catchments. As shown in Figure 2, this particular site has now had its riverbank stabilised though soft engineering techniques of using tree stems and coir matting to overlie the bank face before backfilling this with cobblestones to improve structural strength. This was designed to reduce erosion to improve water quality.
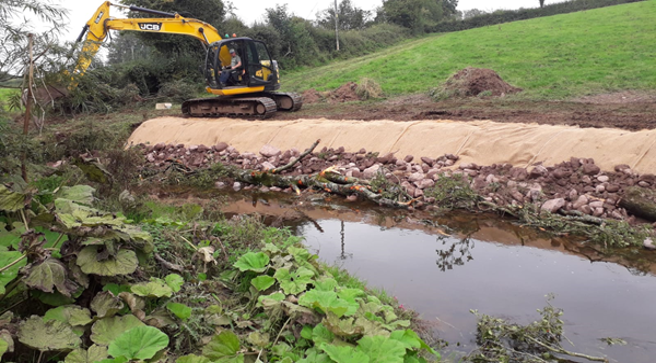
Whilst the calculations obtained for the Blackwater sites are average annual rates and inputs, considerations must be made for the variable and dynamic nature of fluvial systems in terms of alternations of discharge and flow regimes concerning meteorological changes and how this may influence the rate of erosion. Under changing climatological conditions, this is an important factor for consideration, and this particular assessment technique of using LiDAR to quantify annual rates of erosion may be an interesting and novel methodology to monitor any changes in erosion rates under a changing climate.
Bibliography
Cassidy, R., Thomas, I. A., Higgins, A., Bailey, J. S. and Jordan, P. (2019). A carrying capacity framework for soil phosphorus and hydrological sensitivity from farm to catchment scales. Science of the Total Environment, 687, 277-286.
Bastola, S., Murphy, C. and Sweeney, J. (2011). The role of hydrological modelling uncertainties in climate change impact assessments of Irish river catchments. Advances in Water Resources, 34, 5, 562-576.
Rose, R. C. D. and Basher, L. R. (2011). Measurement of river bank and cliff erosion from sequential LIDAR and historical aerial photography. Geomorphology, 126, 1-2, pp. 132-147.
Sharpley, A. N., Daniel, T., Simms, T., Lemunyon, J., Stevens, R. and Parry, R. (2003). Agricultural Phosphorus and Eutrophication (2nd Edition). U.S. Department of Agriculture, Agricultural Research Service, ARS series 149.
Thoma, D. P., Gupta, S. C., Bauer, M. E. and Kirchoff, C. E. (2005). Airborne laser scanning for riverbank erosion assessment. Remote Sensing of Environment, 95, 4, 493-501.
Kommentare